Transition to Large Scale
Nuclear Energy Supply
by
D.A. Meneley, Engineer Emeritus
Atomic Energy of Canada Limited, Mississauga, Ontario
2006 - 2007
president of the Canadian Nuclear Society
2330 Fire Route 26B
Lakefield RR #1 K0L 2H0, Ontario, Canada
Phone: (705) 657-9453
Fax: (705) 657-1914
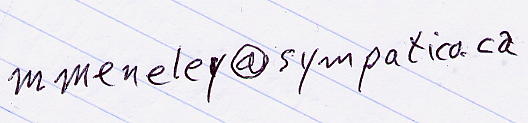
This paper was first presented and published at the 27th Annual
CNS Conference, Toronto, Ontario, June 2006.
It was also published in The Canadian Nuclear Society Bulletin, Vol 27, No. 3.
It is reprinted here with the permission of the author and the CNS. Copyright,
Canadian Nuclear Society, 2006.
Abstract
We can expect to see the peak of world oil production very
soon. Some say that we can see that peak now in our rear-view mirrors as we
drive into an oil-poor future. Natural gas already is in short supply in North
America. Nuclear energy must make up the lion’s share of the world’s energy
deficit.
This paper examines, in very general terms, the implications of today’s shifting
prospects for nuclear energy, as it exists today, and how those prospects might
develop in the future. The time span under consideration is the remainder of the
21st century.
Introduction
During the working lives of many professionals active today, the nuclear power
industry has hovered on the brink of extinction. Will people accept the
technology? Who will buy the next plant, and if they buy, at what price? Will
the competition get the job? Will government support pre-commercial product
development? We all have asked these questions. Now, the questions are
changing.
Oil supply analysts[1] agree that world oil production must decline at some time
during the 21st century. Huge imports of natural gas to North America will be
needed in the near future. Which projection is correct? Will new discoveries
solve the problem? Will demand moderate as prices increase? Where can we find
alternative energy sources on a massive scale?
Obviously, when looking at a 100-year time frame it makes no sense to propose
the solution to energy supply questions. Rather than make such an attempt, the
author has chosen to follow published projections as far as they go and then to
make reasonable guesses at a series of development steps that can be taken to
reach a defined goal at the end of this century. To some extent this process is
based on a recent IAEA symposium[2] with the declared goal of looking at the
future of the world nuclear industry.
History
Uranium was recognized as a vast potential source of energy from the first days
after the discovery of nuclear fission[3]. Leo Szilard[4] quickly recognized its
potential for both good and bad purposes.
When R&D for the nuclear-electric industry began in the 1940’s and 50’s one of
the main concerns was the potential shortage of uranium fuel. At the time,
exploration for uranium was limited and only low ore concentrations had been
found. This apparent resource shortage led to intensive work on fast-spectrum
reactors; indeed, the first-ever electricity production from uranium was from a
fast reactor-- the Experimental Breeder Reactor (EBR1) in Idaho. During my own
time of research at Argonne National Laboratory, a great deal of work centered
around calculation of the breeding ratio – a figure indicative of the amount of
fresh fissile material that can be produced by a fast reactor power plant.
Dr. W.B. Lewis[5] was deeply involved with the uranium supply question, both
nationally and internationally. Both uranium and thorium fuel cycles were
studied in detail. The latter element, of course, suffers from a lack of any
naturally occurring fissile isotope so that uranium must provide the initial
fuel supply. Atomic Energy of Canada (AECL) under Lewis’ direction studied
several other means of producing fissile isotopes, notably by accelerator-driven spallation reactions capable of producing large numbers of neutrons for
subsequent capture in the abundant fertile isotopes Thorium-232 and Uranium-238.
Successful uranium exploration in the 1960’s and 70’s greatly increased known
uranium reserves. Large ore deposits were found in Canada, the Soviet Union and
Australia, along with important quantities in several other countries. The
total amount of uranium in the earth’s crust is immense – and yet we do not know
how much recoverable ore might be found in the future. At the present time the
supply-demand pendulum appears to be swinging back toward higher prices, as the
demand for fresh nuclear fuel increases.
This paper is not a “hard-and-fast” plan for the future. Further, it makes no
pretence toward arguing that this future conceptual plan is the only one
possible, or even that it may be the preferred plan. The objective of this
paper is to illustrate some of the opportunities along with a few of the hurdles
that must be passed along the way, in order to realize the vision of a secure
and sustainable energy supply in the future world.
The Need for Nuclear Energy
Today’s world depends heavily on petroleum, both oil and natural gas. It is
still an open question as to what energy source or sources can and will take
over the burden once this resource is depleted. Many options are available that
can contribute to the solution, but it appears that nuclear energy must be a
major contributor[6].
There has been much talk in recent years about the “Hubbert’s Peak”[7] of world
oil production. According to that model, once the peak has been reached one
should expect that only one half of the total resource remains to be found. Now
that prices have been high and rising for 5-10 years and yet production has been
decreasing over the same period, we can safely conclude that we have passed the
peak of world production.
Conservation, along with a number of alternate energy supply options, has been
studied for a number of years with limited success. It has slowly become
obvious that nuclear energy is the only resource available today that could take
over a large fraction of the world demand for oil and gas, and yet remain
neither capacity nor resource limited – that is, to be “inexhaustible” or
“renewable”. There is enough accessible uranium to supply the total present-day
demands of humanity for at least several thousand years[8].
The question “How much energy does the world need?” is the most important and
most difficult question of all, and well beyond this author’s capability to
estimate. As a scale comparison, the total world energy demand of the World
Energy Council (WEC) ‘middle course’ scenario in 2050 is given as about 400
million barrels per day of oil equivalent[9]. The WEC scenarios show a slowly
decreasing role for coal, a large role for natural gas, and a steadily
increasing contribution from solar and biomass at that time. As noted briefly
in this paper there is a real possibility for using coal or biomass to
manufacture synthetic transportation fuels by adding hydrogen. Nuclear energy
can be used in this way to produce fuels that are fully compatible with today’s
transportation, heating, and other industrial systems.
Since coal and natural gas do not seem to be scarce in a worldwide context, this
work concentrates on substitution of nuclear energy for oil – a commodity that
is rapidly becoming scarce.
General Industrial Plan[10, 11]
Presumed world oil demand in this study is shown in Figure 1. Up to 2030, the
estimate is taken from the 2005 International Energy Agency (IEA) projection[12].
Beyond that time demand is assumed, arbitrarily, to flatten out at 140 million
barrels per day.
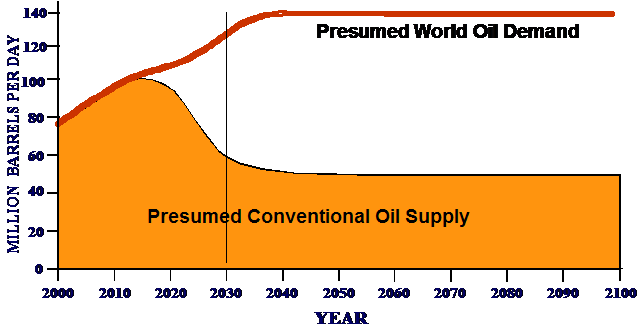
Figure 1: Presumed oil supply and demand vs. time
The supply curve for conventional oil also is taken from IEA figures up to
2030, without non-conventional supply and new discoveries. After that time
supply is assumed to flatten out at 50 million barrels per day, or about half of
the peak production in 2005. (Note: There is no presumption that the long-term
“Supply” curve in Figure 1 is a prediction of what will happen. The essence of
the question is the timing of the expected supply deficit and the fact that it
will continue indefinitely into the future.) Some fraction of the deficit
between conventional supply and total supply will be filled from other sources
such as conservation, introduction of hybrid vehicles, new oil discoveries,
wind, solar, and so on. It is important to note that the particular timing of
peak world oil production is quite unimportant. It is necessary only to agree
that there will be a peak of production, at some future time. In other words it
is necessary only to accept the fact that recoverable oil is a finite commodity
on earth.
So, what should we do about it? There have been many studies conducted, and
many proposals put forward. The present-day situation has recently been
summarized[6, 8]. These modern assessments differ little from that described in
the International Institute for Applied Systems Analysis (IIASA) study carried
out more than 20 years earlier[13]. The main change since the IIASA study is that
the needed replacement for fossil fuels is now urgent. Nuclear energy using
uranium offers the only practical answer for filling in a major part of the gap
between supply and demand shown in Figure 1. Even then, the enormous scale of
the replacement task cannot be over-emphasized. This is not to belittle
contributions of other renewable resources and conservation. The statement is
meant only to emphasize the central role of nuclear energy in any sound plan,
regardless of what other partial solutions are adopted.
Substitution of nuclear fuels for fossil fuels in the supply of primary energy
is not a simple task. For instance, transportation requires a portable fuel of
high energy density and low weight – that is, if we choose to mimic today’s
pattern of transportation. The refining and distribution of fossil fuels now
embodies a massive infrastructure that pervades nearly every corner of North
American society. A similar complex infrastructure is seen in the electricity
distribution system – it is difficult to scan the horizon in any industrial
nation today without seeing some evidence of this second system. Could
they/should they be combined in some way? This might increase efficiency, but
might at the same time increase the system’s vulnerability.
Substitution of nuclear for fossil supply can be approached in different ways.
It is possible to expand the electrical distribution system and then to provide
local service either for battery-powered vehicles or for some form of hybrid.
The next question is whether or not batteries can be developed that can match
the excellent characteristics of gasoline- and diesel-powered systems. Today’s
answer seems to be “not yet” though there is hope that this will be possible
soon[14].
A second method of substitution is to produce an intermediate energy carrier[15]
such as hydrogen that can be utilized in different ways such as local
night-to-day storage for peak leveling, in fuel cells, or as feedstock for
manufacture of hydrocarbons. Hydrocarbon production is practiced today in South
Africa[16], using an improved process relative to the basic method developed in
Germany in the 1920’s[17]. Production of hydrogen can be done either by direct
electrolysis or by a number of alternate chemical processes. Hydrogen is
particularly difficult to store and transport; local generation of hydrogen from
electricity is being considered as an alternative to central generation and
pipeline distribution. No single method has yet emerged as being superior to
others.
Strategies for Installation of Large-Scale Nuclear Supplies
Even though there is strong evidence that the long-term nuclear future must be
based on fast reactor technology, almost all nuclear plants operating in the
world today are powered by thermal reactors. As a result we must consider the
necessary steps in a transition from today’s technologies to those appropriate
for the long-term future in which the predominant source of primary energy is
nuclear fission.
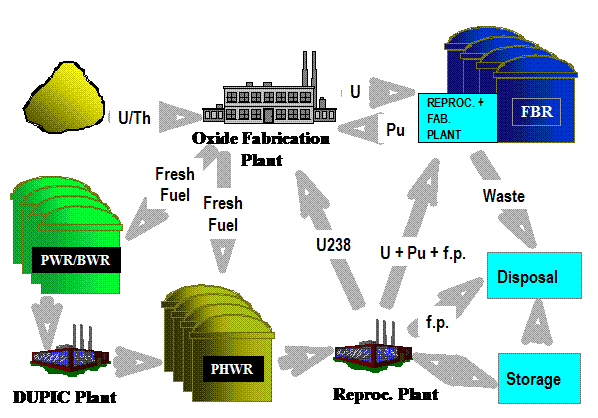
Figure 2 - Conceptual Arrangement of a Nuclear Energy Park
Figure 2 is adapted from an earlier paper[18]. It is a concept
sketch of an “energy park”, as several authors have discussed over the past
decades. This version of the energy park concept includes all components
essential to production, from fuel input to waste disposal. These components
may of course, be either dispersed or concentrated. It is useful to think of
them as being co-located on an “energy island”, either figurative or literal.
An actual island might be preferred if security and safeguards are assumed to be
dominant factors in this postulated future scenario.
Figure 2 can be considered as a “target scenario”; that is, a future energy
system toward which we could now aim, while recognizing that its actuality will
be achieved only after several steps and stages collectively requiring several
decades for implementation. The system includes fuel recycle facilities such as
electrochemical reprocessing[19] or direct use of PWR fuel in CANDU (DUPIC)[21].
An energy park such as this brings with it several advantages. First it is
large, so that costs of perimeter security are distributed over a number of
profit centers. In addition, this large scale permits the establishment of a
large staff with diverse technical skills, and a revenue base capable of
supporting effective waste management systems such as zeolite trapping of
radioactive noble gases. Energy from such a facility may be distributed by
electrical power lines, via tankers in the case of synthetic
fuel production, or in the form of solid products such as industrial chemicals
or fertilizer.
Given the high probability of ongoing supply crises in world oil and gas supply
during the next couple of decades it is obvious that the only nuclear
technologies ready for immediate deployment in large numbers are the pressurized
water reactor (PWR), the boiling water reactor (BWR), and the pressurized heavy
water reactor (PHWR). All of these reactor types produce electricity at
mutually competitive prices. Further, if the authorities that must buy these
power plants are conservative in their choice of appropriate technology, these
plants are likely to be the same or very similar to plants operating today. As
decades pass, new improved designs based on similar technologies will be chosen
more frequently as their advantages come to be more strongly assured.
Recognizing that hundreds of thermal reactor plants will be operating before a
significant shift toward fast-reactor powered plants comes onto the market, it
may be possible to choose some variant of thermal reactor that would make the
later transition easier. From the point of view of fuel cycle sustainability,
the most important thermal-reactor characteristic is the amount of electricity
that can be produced per unit of natural uranium required to supply fuel to the
plant. By this measure, the PHWR-CANDU is clearly superior.
Table I – Energy Output per Megagram of Uranium Mined |
|
MWy(e)/Mg |
Enriched U in PWR, BWR |
4.61 |
Pu Recycle in PWR, BWR |
5.41 |
DUPIC (PHWR-CANDU) |
6.37 |
Natural U in PHWR-CANDU |
6.37 |
1.2% Enriched U in PHWR-CANDU |
8.77 |
Table I shows the energy produced per Megagram of mined uranium18. It shows that
a fleet of PHWR reactors can produce 30-60% more electricity than can the same
number of PWRs, from a given amount of mined uranium. Fuel discharged from a
once-through cycle in a PHWR can be sent to a reprocessing plant to extract
uranium 238 as well as some high-absorption fission products and produce fresh,
recycled fuel.
Another advantage of the PHWR is illustrated by considering a simple equilibrium
steady-state ratio of thermal reactors to fast reactors in a combined system
where fast reactors provide fissile isotopes to thermal reactors. Wade[10] shows
that this ratio is given by the equation
Number of thermal units/Number of fast units ≈ (BR-1)/(1-CR)
The approximate values of conversion ratio (CR) and breeding ratio (BR) are: FBR=1.4,
PWR, BWR=0.6, PHWR=0.8, PHWR(Th)=0.95.
Table II – Equilibrium
Ratio of Thermal to Fast Reactors – Equal Energy Output |
Reactor Type |
Thermal/Fast Ratio |
PWR, BWR |
1.0 |
PHWR-CANDU |
2.0 |
PHWR-CANDU (Th)* |
5.0 |
* Seed-blanket fuelling with Pu-U driver fuel
and Thoria blanket fuel. |
Table II lists the consequent ratio of
thermal to fast reactor plants for each thermal reactor system.
When the first fast reactor begins operation the actual ratio will be much
larger than this equilibrium value. As more fast reactors are started up the
actual ratio will decrease with time, toward this equilibrium. New fast
reactors will be fuelled from processed PWR, BWR and PHWR materials along with
excess plutonium recovered from operating metal-fuelled fast reactors via
electrochemical (pyrometallurgical) processing[19].
Success of the PHWR (Th) system depends entirely on the presumed capability for
reprocessing discharged Th-U233 fuel and utilizing the bred uranium-233 in fast
reactors. It must be recognized, however, that the achievable maximum breeding
ratio with Th-U fuel in a fast reactor is somewhat lower than is achievable in a
uranium-plutonium cycle. For simplicity, for this discussion we can lump
uranium and thorium together as fuels, because in our integrated system all
isotopes of the thorium and uranium series will be totally consumed at some step
in the cycle.
A serious restriction on the growth rate of the integrated nuclear generating
system arises from the shortest-achievable value of compound doubling time of
FBR reactors – which is about ten years. This figure sets an upper limit (about
5 percent per year) on the rate of increase of fast-reactor-powered nuclear
stations, even if all the fuel produced is recycled into new units. Of course,
if other recycled fuel is available from thermal reactors, this rate can be
increased so long as such recycled materials are available. Y.I. Chang[11] gives
an excellent summary of these fuel-supply limitations. Clearly, the high cost
of fuel would soon limit any system using only thermal reactors because they can
utilize only about one percent of the potential energy in mined uranium.
Technologies – What more is needed?
The predominant area of need for new industrial capacity relates to fuel
recycle. The technology of the Integral Fast Reactor (IFR) is well
established19, and a viable commercial plant design is in hand[20]. Pyroprocessing is known to work at the bench scale but still must be
demonstrated on a larger scale before qualifying fully as a commercial process.
Recycling of used fuel from thermal reactors first requires extraction of
uranium 238; separation of some neutron-absorbing rare earth elements via an
oxidation-reduction process known as OREOX21 could be used to improve the
recycled product. The product then consists of transuranic elements and some
fission products. This mixture is excellent as a fuel for fast reactors.
In a combined fuel cycle system such as this, the last step of the cycle will be
located in metal-fuelled fast reactors with integral reprocessing facilities.
During this final step (which will include a few recycles within each plant’s
reprocessing facility), essentially 100% of the transuranic elements will
undergo fission. The processing facility output will, as a result, consist
almost totally of fission products – an important feature of this fuel cycle,
because it reduces the necessary time of waste isolation to five hundred years
or less. This eliminates the need for a special long-term waste repository –
final waste disposal probably can be located directly under the energy park, in
a deep borehole.
Within a static or slowly growing fleet of power plants, provided that more fast
reactor units are operating than the equilibrium number indicated above, the
configuration of some reactors can be adjusted to reduce the amount of plutonium
produced. However, in a growing fleet with fewer than the equilibrium number of
fast reactors operating, the total inventory of fissile material will decrease
steadily unless more is added from an external source such as reprocessed LWR
fuel or newly mined uranium. The total quantity of the first is known, and
limited. The quantity of uranium available is flexible and depends on the price
that buyers are willing to pay. This ‘demand price’ can be extremely high in an
equilibrium system of thermal and fast reactors because of the enormous amount
of energy that can be extracted from each unit of uranium8.
Clearly, in a system including a supra-equilibrium number of fast reactors every
fissile atom has a high value because it represents an opening toward extraction
of 100% of the potential energy in mined uranium. Mining, even in very
low-grade deposits, still benefits from a strong economic incentive. Uranium
enrichment may be required in times of rapid energy demand growth; even in that
situation uranium tails may still have positive economic value because of their
eventual application as blanket materials in fast reactors.
*
In summary, the major new components yet to be established are:
*
A large and growing fleet of fast-reactor-powered nuclear plants
*
An integral pyroprocessing/fabrication plant for metallic fuel at each unit
*
Reprocessing plants for recycling LWR and HWR fuels
*
Fabrication plants for fuel recycled from LWR and HWR units
Means other than isotopic separation may be feasible for sustaining the fissile
isotope inventory in times of rapid electricity demand growth.
Accelerator-driven spallation is one such possibility[5]; a fusion-fission hybrid
concept also has been proposed[22].
Power Plant Sites and Characteristics
The large scale of nuclear production facilities that may be required might
influence our consideration of options. To get an impression of the scale
involved, the total output of about 630 one-gigawatt-electric (GWe) nuclear
units would be required to replace the daily average energy released by burning
gasoline in North America today.
Today’s worldwide fleet of nuclear plants comprises about 430 units that in
total generate less than 400 Gwe. These plants are accommodated on more or less
conventional sites. However, if plants with a projected total capacity of
5,000-10,000 Gwe are to be installed over the next decades the choice of plant
sites will become a substantial problem. Very large sites (up to ~50 Gwe each)
will be preferred. These sites would be large enough to sustain a broad array
of technical expertise as well as fuel cycle support and security facilities.
Comprehensive security systems would be a necessary and affordable feature.
Recycling, waste management and disposal systems would be included. Secondary
industries such as hydrogen production and synthesis of liquid transportation
fuels could be established on the same site. Distribution of energy from such
sites will require a large infrastructure – not unlike that surrounding large
oil and gas production centers such as those in the Persian Gulf. Manufacture of
satellite power systems[10,10a] also may be undertaken. These satellite systems
can be considered as a further means of distributing potential nuclear energy
from these large central sites.
Site Facilities
An energy center should be built step by step, according to a broad but
adaptable overall plan. The Bruce site on Lake Huron provides a good example of
how such a complex might begin[23]. The site now includes about 7 Gwe of
generation plus a number of support facilities. Some years ago, a conceptual
plan[24] was put forward for a multi-stage energy cascade system adjacent to the
site. On-site used fuel storage facilities are already in place. Heavy water
production plants that were a feature of the site in earlier days are shut down.
The next step of site development could be addition of more generation capacity;
if this step is taken in the near future a good choice will be CANDU reactor
units, either of the type now operating or the new Advanced CANDU Reactor (ACR) type. Later on, integral
fast reactors might be added as a first move toward a system with a closed fuel
cycle. These reactors could utilize the used CANDU fuel now stored on site,
given the addition of a processing plant. (There is already sufficient used
fuel on site to power an integrated generation complex of ~15 Gwe for several
hundred years.) A U238 extraction plant could upgrade this fuel and supply the
first charge to each fast reactor as well as recycling mixed-oxide fuel to
onsite CANDU units. Depending on the rate of capacity buildup it may be
necessary to supply a limited amount of enriched uranium or separated plutonium
to the site from external sources.
Depending on circumstances in the external market, management of a mature site
such as this might choose to install a number of fast reactors above its
equilibrium level, and sell plutonium-bearing fuel to other similar sites still
under development under strict international control. The core and radial
blanket configuration of each fast reactor can be adjusted to regulate amount of
excess plutonium produced on the site.
Fuel Supplies
Fuel requirements are very small at an integrated fast-thermal reactor site.
Basically, the amount of fresh uranium needed to sustain a metal-fuelled fast
reactor using integral pyroprocessing, located on an ocean site, will be less
than the amount of uranium dissolved in the seawater required to cool its
turbine condenser (seawater has a dissolved uranium concentration of 3 parts per
billion.) In other words, only about 1/100th of the amount of fresh uranium now
required per operating megawatt of capacity will be sufficient to sustain
generation. The Bruce Energy Centre is a fresh water site; this illustration
simply indicates the very small quantity of uranium needed to sustain such an
integrated system.
World Nuclear System by 2100
It is possible to imagine a world energy supply system operating in about 100
years. That system could consist of 10,000 Gwe of generation and associated
peripheral systems, located on 100-200 sites worldwide. Some of these sites
might be dedicated to production of synthetic petroleum liquid and gas as well
as a wide range of other industrial processes. At the low-temperature end of
production cascades one might find food-related installations such as fertilizer
production and fish farming. This network of large energy parks might be
interspersed with smaller, independent installations using sealed “nuclear
battery” power systems[10]. Reference 10a outlines an extension of this concept.
There is enough uranium available for human use so that this large-scale world
energy supply can be sustained for at least several thousand years[8].
Conclusions
This “Blue Sky’ concept paper shows that a sustainable nuclear system can be
built up, step by step, from components and systems already proven and available
today, and augmented by simple extensions of proven concepts – all well within
the realm of known technology. Further work is required to guide the selection
of reactor types and fuel cycle facilities during development of energy parks.
Several prototype facilities must be established before commercial viability can
be proven.
References
1. M.R. Simmons, “Twilight in the Desert”, John Wiley & Sons, (2005),
ISBN-13: 978-0-471-73876-3
2. N. Oi, L. Wedekind, International Atomic Energy Agency (IAEA), Key Issue
Paper #3, Proceedings of the International Symposium on Nuclear Fuel Cycle and
Reactor Strategies: Adjusting to New Realities, (June 1997). URL: <http://www.iaea.org/Publications/Magazines/Bulletin/Bull401/article2a.html>
3. L. Meitner, O.R. Frisch, Nature (February 11, 1939)
4. R. Rhodes, “The Making of the Atomic Bomb”, p. 27-28, Simon & Schuster,
(1986), ISBN 0-671-44133-7
5. W.B. Lewis, “The Intense Neutron Generator and Future Factory Type Ion
Accelerators”, AECL, Chalk River Nuclear Laboratories, Report AECL‑3190.
Presented to IEEE-GNS 15th Annual Meeting, Montreal, (October 1968)
6. C.E. Till, “Plentiful Energy, the IFR Story, and Related Matters.” THE
REPUBLIC News and Issues Magazine, (June-September 2005).
7. M.K. Hubbert, “Nuclear Energy and the Fossil Fuels”, American Petroleum
Institute, San Antonio, (March 1956). URL: <http://www.hubbertpeak.com/hubbert/1956/1956.pdf>
8. H. D. Lightfoot, W. Mannheimer, D.A. Meneley, D. Pendergast, G. S.
Stanford, “Nuclear Fission Energy is Inexhaustible”, Proceedings of the Climate
Change Technology Conference, Engineering Institute of Canada, Ottawa (May 2006)
9. World Energy Council, “Global Energy Scenarios to 2050 and Beyond”,
(2005), URL <http://www.worldenergy.org/wec-geis/edc/scenario.asp>
10. D.C. Wade et al., STAR H2: The Secure Transportable Autonomous Reactor for
Hydrogen (Electricity and Potable Water) Production. NERI Project No. 2000-0060,
Argonne National Laboratory, (2000) URL: www.hydrogen.anl.gov/pdfs/STAR-H2summary.pdf
10a Global Nuclear Energy Partnership, URL: www.gnep.energy.gov/
11. Y.I. Chang, “Advanced Fast Reactor: A Next-Generation Nuclear Energy
Concept”, Forum on Physics & Society, American Physical Society, (April 2002),
URL: <www.aps.org/units/fps/newsletters/2002/april/a1ap02.cfm>
12. “World Energy Outlook 2005”, OECD/International Energy Agency, Paris,
(2005)
13. W. Haefele – Program Leader, “Energy in a Finite World – A Global Systems
Analysis”, Harper & Rowe, (1981). ISBN 0-88410-642-X
14. “Renewable Energy use in Transportation”, Proceedings of Conference, Dr.
Eric Bibeau (Ed.), University of Manitoba, (June 20, 2005). URL: <http://www.umanitoba.ca/engineering/mech_and_ind/prof/bibeau/cec/cec.html>
15. “Nuclear Production of Hydrogen – Technologies and Perspectives for Global
Deployment”, M. Hori, J. Spitalnik, (Ed.), International Nuclear Societies
Council, (Nov 2004), ISBN: 0-89448-570-9
16. Historical Overview of the South African Chemical Industry: 1896 – 2002,
URL: http://www.caia.co.za/chsahs03.htm
17. Dr. Franz Fischer, “The Conversion of Coal into Oils”, Ernst Benn Ltd.,
(1925), and “The Fischer-Tropsch Synthesis”, Robert Bernard Anderson, Academic
Press, (1984)
18. D.A. Meneley, A.R. Dastur, P.J. Fehrenbach, K.H. Talbot, “Synergistic
Nuclear Fuel Cycles of the Future”, Global ‘95, Versailles, France, (September
1995)
19. “The Technology of the Integral Fast Reactor and its Associated Fuel
Cycle”, T.D. Beynon, D.J. Dudziak, (Ed.), W.H. Hannum, (Guest Ed.), Progress in
Nuclear Energy 31, Number 1/2, (1997)
20. A.E. Dubberly, “S-Prism High Burnup Metal Fuel Core Design”, Proceedings of
ICAPP ’03, May 4-7, Cordoba, (May 2003)
21. P.G. Boczar, “CANDU Fuel Cycle Vision”, IAEA Technical Committee Meeting on
Fuel Cycle Options for LWRs and HWRs, Victoria, 622-I3-TC-529.6, (April 28-May
1, 1998)
22. W. Manheimer, “Back to the Future: The Historical, Scientific, Naval and
Environmental Case for Fission Fusion”, Fusion Technology, Vol. 36, (July 1999)
23. D.A. Meneley, R.B. Duffey, D.R. Pendergast, “CANDU Co-Generation
Opportunities”, IAEA Technical Committee Meeting “Prospects of Non-Electrical
Applications of Nuclear Energy”, Beijing, (April 1999)
24. G.M. Gurbin and K.H. Talbot, “Nuclear Hydrogen - Cogeneration and the
Transitional Pathway of Sustainable Development”, 9th Pacific Basin Nuclear
Conference, Sydney, Australia, (May 1994)
BACK